激光尾波场电子加速及新型辐射源(特邀)
下载: 1008次创刊五十周年特邀【增强内容出版】
受益于超短超强激光技术的持续迅猛发展,飞秒强激光为人类提供了全新的实验手段与极端的物理条件,使激光物质相互作用进入到一个极端非线性的强场超快新范畴,催生了大量新原理、新现象,推动了技术变革。飞秒强激光驱动的等离子体尾波场加速原理是一种具有超高加速梯度的粒子加速新原理,该技术的加速梯度可达100 GV/m,相比于传统射频加速器提高了3个数量级以上,可在厘米量级的加速长度内获得GeV量级的高品质高能电子束,极大地降低了加速器的成本,为发展新一代粒子加速技术和新型超快辐射源提供了新机遇和新途径。从飞秒强激光驱动等离子体尾波场中的电子注入、能量啁啾控制和高品质电子束产生以及基于高品质电子束的betatron X射线辐射、高能伽马射线和小型化自由电子激光这几个方面介绍了激光等离子体尾波场电子加速的若干主要研究进展,并对未来进行了展望。
The rapid development of ultra-intense and ultra-short lasers has provided unprecedented new experimental methods and extreme physical conditions, made it possible to reach new frontiers of ultra-fast and intense interactions between lasers and matter, and given birth to a large number of new principles, new phenomena, and revolutionary techniques. Plasma-based acceleration driven by an ultra-intense and ultra-short laser may contribute to the emergence of new particle-acceleration technologies and generation of novel ultra-fast radiation sources. These novel particle and radiation sources can provide new means and opportunities for frontier interdisciplinary studies in areas such as high-energy particle physics, nuclear photonics, materials science, and biomedicine, making it a hot spot and emerging field on the world scientific and technological frontiers.
The accelerating electric field of a laser-driven plasma wakefiled can reach 100 GV/m, which is more than three orders of magnitude higher than that of a traditional electron accelerator. A high-energy GeV electron beam can thus be produced over a centimeter-scale acceleration length, thus greatly reducing the scale and cost of the accelerator. The electron beams produced via laser wakefield acceleration also have the advantages of an ultrashort pulse duration and inherent high-precision synchronization with the driving laser. In addition, by designing an appropriate and effective scheme, the electron-injection and acceleration processes can be optimized to produce high-quality and high-energy ultrafast electron sources with ultrahigh brightness comparable to that from a traditional accelerator.
Laser-wakefield-driven electron beams can be used as low-cost and desktop femtosecond radiation sources such as for betatron X-ray radiation, inverse Compton scattering, bremsstrahlung radiation, and undulator radiation. These novel radiation sources usually have high brightness, good collimation, a femtosecond pulse duration, and energy tunability, covering a wide spectral range from extreme ultraviolet to gamma rays. Therefore, research in this area is occurring around the world, and this is an important research topic for high-field laser physics and new accelerators. Such laser wakefield acceleration and novel radiation sources are thus of great scientific significance for the development and application of synchrotron radiation, free electron lasers, and high-energy particle physics.
After nearly 20 years of development, great progress has been made in both experimental and theoretical studies on laser-driven plasma acceleration. It is now transitioning from laser acceleration to laser accelerators. On one hand, the energy gain of laser wakefield electron acceleration has been significantly extended to 7.8 GeV. On the other hand, the specific qualities of the accelerated electron beams produced via laser wakefield acceleration, such as the energy spread, divergence, emittance, and stability of the electron beam, are also being optimized to a great extent. However, the comprehensive performance has to meet higher requirements for practical application, and there are still many key scientific issues and technical difficulties that need to be further explored and solved in the future. In particular, the energy spread of the electron beam is usually on the order of several percent, and such a large energy spread has greatly hindered its practical application. In order to obtain more stable and brighter high-energy electron beams, the electron injection and acceleration in the plasma wakefield should be accurately controlled and optimized to minimize the energy spread and divergence, which can also improve the application performance of novel radiation sources. Therefore, the basic principles and parameter characteristics of a plasma wakefield driven by a femtosecond intense laser are first briefly introduced. The mechanisms and characteristics of different electron injection methods are then analyzed and compared (Table 1). Second, based on the research results and progress made by our group in recent years, the schemes and technologies for exploring energy chirp control in a plasma wakefield with a structured plasma profile are summarized and analyzed in relation to the generation of ultrahigh-brightness electron beams with an ultralow energy spread at a per-mille level (Fig.5). Third, we discuss how these high-quality electron beams are used to produce novel radiation sources and greatly improve their application performance, including enhanced betatron X-ray radiation (Fig.9), quasi-monoenergetic all-optical self-synchronized Compton scattering γ‑rays (Fig.18), and free-electron lasing in an undulator (Fig.22). Some of the progress in other related frontier research fields is also discussed in relation to laser wakefield electron acceleration and novel radiation source generation. Finally, the prospects for a laser wakefield electron accelerator and its further practical applications are outlined.
A high-quality electron beam source and novel radiation source based on laser wakefield acceleration have the advantages of a compact size, easy tuning, small source size, femtosecond pulse duration, high brightness, good collimation, and high-precision synchronization control, which can provide new methods and tools for frontier interdisciplinary research such as high-energy particle physics, nuclear photonics, materials science, and biomedicine. Although significant progress has been made in the past decade in improving the quality of an electron beam such as its energy spread and six-dimensional brightness, the wakefield accelerator is still in a very early phase in view of the energy spread and stability of the electron beam, especially for electron beams with energy levels below 100 MeV or above 1 GeV, when compared with traditional accelerators. This dilemma is mainly limited by the scalability and stability of the existing schemes. The key issue or challenge facing the wakefield acceleration community is to devise more effective schemes to generate electron beams with an ultralow energy spread (0.1%‒0.01%), ultralow emittance (~1 μm·mrad), high repetition rate, and stability. Benefiting from the rapid and continuous development of ultrashort pulse laser technology in terms of the repetition rate, waveform control, and stability of the high-power laser, it is believed that the qualities and brightness of these high-energy ultrafast electron beams will be further improved by advancing the existing schemes, which will further facilitate the development of novel radiation sources. All these advances will greatly promote the continuous development of high-quality laser wakefield electron accelerators and their practical applications in the years to come.
1 引言
受益于啁啾脉冲放大技术[1],超短超强激光技术在过去30多年里得到了快速发展,高功率脉冲激光的峰值功率已经提高了3~5个量级,从太瓦(TW,1012 W)级发展到10拍瓦(PW,1015 W)级[2],激光的脉宽越来越短,从皮秒级发展到几十飞秒(fs,10-15 s)级,甚至到几个飞秒的周期量级。超短超强激光技术为人类提供了全新的实验手段与极端物理条件,使激光物质的相互作用进入到一个极端非线性的强场超快新范畴,催生了很多新原理、新现象,推动了相关技术变革[3]。如:采用飞秒强激光驱动等离子体尾波场加速电子,可以获得时间宽度短至数飞秒的高能量电子束源[4-5];利用光压加速、靶后鞘层加速以及静电激波加速获得高能离子束[6-8];利用飞秒强激光驱动气体、固体高次谐波等极端非线性过程获得更短脉冲,如阿秒级的相干X射线光源等[9-13]。
1979年,Tajima和Dawson[4]提出了一种利用激光驱动等离子体尾波场加速电子的新概念,但是受当时超短脉冲激光技术的限制,激光尾波场电子加速的相关研究一直没有获得实质性进展。直到2004年,太瓦级飞秒强激光的出现使得激光等离子体尾波场电子加速有了突破性进展,获得了百MeV级的准单能电子束[14-16],其被称为“梦之束”。该技术的加速梯度相比于传统的射频电子直线加速器提高了3个量级以上,飞秒强激光驱动的尾波场电子加速提供了一种全新的小型化电子加速新途径,极大地缩短了加速距离,降低了加速器的成本,同时也避免了初始加速阶段电子束纵向和横向的展宽效应,从而获得横向尺度和脉冲宽度非常小的高性能高能电子束。相比于传统射频加速器,激光加速的电子束天然具有脉冲短(数飞秒甚至阿秒级)、发射度小、与驱动激光同步精度高等显著优点,对同步辐射、自由电子激光及高能粒子物理等领域的发展和应用具有重大科学意义。
自2004年以来,激光尾波场电子加速以及基于激光加速电子的新型辐射源一直是强场激光物理领域的研究热点和难点。经过近20年的发展,激光等离子体加速取得了长足的进步,特别是激光驱动等离子体尾波场电子加速正处于从激光加速向激光加速器跨越的重要关口。一方面,激光尾波场加速电子的能量增益不断提高,正在向10 GeV的目标迈进,这样高能量的电子束加速需要利用大型的飞秒拍瓦级甚至10拍瓦级激光装置。2019年,Gonsalves等[17]利用拍瓦级激光在放电毛细管波导中驱动等离子体尾波场加速电子,在近20 cm长的等离子体通道中获得了峰值能量为7.8 GeV的高能电子束。另一方面,激光尾波场加速电子束的性能(如电子束的能散度、发射度、稳定性等)也在不断改善。2016年,Wang等[18]通过实验获得了能散度小于1%、发射度小于1 μm的数百MeV量级的高能电子束。这样高品质的电子束可以用来发展各种超快X射线、伽马射线辐射源、台式化自由电子激光以及电子衍射成像技术等[19-25],并为材料、超快化学、生命科学等前沿交叉基础研究提供了新手段和新机遇。本文主要从飞秒强激光驱动的等离子体尾波场中的高品质电子加速以及新型辐射源如betatron X射线辐射、逆康普顿伽马射线和台式化自由电子激光这几个方面介绍了激光等离子体尾波场电子加速的若干主要研究进展。
2 激光等离子体尾波场电子加速中的电子注入
当一束激光或者电子束在等离子体中传播时,激光束的有质动力或者电子束的库仑力会将等离子体中的电子向外排开,由于离子的质量远大于电子的质量,其响应时间远长于电子的响应时间,故可以认为离子是相对静止的,从而形成一个很强的电荷分离场。被排开的电子在激光的有质动力和电荷分离场的作用下在平衡位置附近产生周期性的电荷振荡,形成等离子体尾波[5,26]。激光激发的等离子体尾波场以接近光速的速度随激光向前传播,其相速度(vp)约等于驱动激光在等离子体中传播的群速度(vg),即vp≈vg=
表 1. 激光尾波场电子加速实验中不同电子注入方案及性能参数对比
Table 1. Comparison of different electron injection schemes and performance parameters in laser wakefield electron acceleration experiments
|
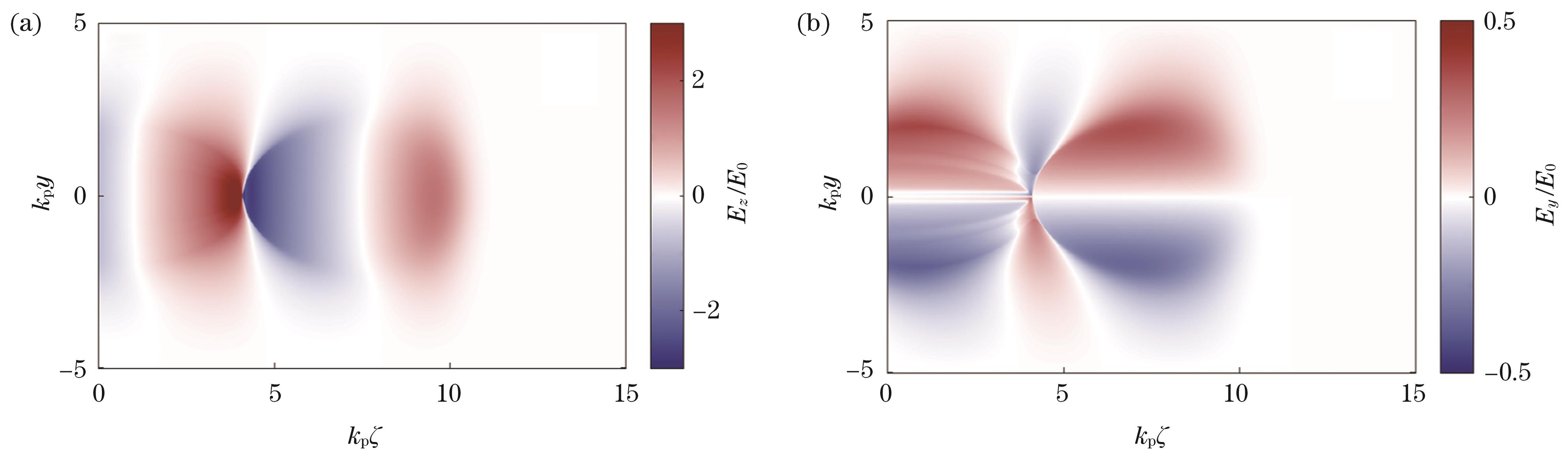
图 2. 空泡中电子所受到的电场。(a)纵向电场;(b)横向电场
Fig. 2. Electric field on electron inside bubble. (a) Longitudinal electric field; (b) transverse electric field
激光激发尾波场中的纵向电场强度依赖于n0和a0,且等离子体可维持电场强度超过E0的等离子体波[5],E0=mecωp/e≈96
在激光驱动等离子体尾波场的过程中,电子的初始速度如果达不到尾波场的相速度,电子会相对于激光尾波场向后滑移而无法被尾波场捕获,因此只有满足一定初始条件的电子才能被尾波场捕获并加速。因此,与传统射频加速器一样,在激光尾波场加速过程中,电子束的注入控制是一个非常重要的关键技术,主要包括自注入、对撞注入、电离注入和密度梯度注入等注入方案。
自注入指的是通过激发强非线性的等离子体波来实现对等离子体背景电子的捕获和注入[30-32]。原则上,当电子的初始纵向速度大于电子所在位置的等离子体波的相速度时,电子就会被注入到空泡内。当尾波振幅增强、背景电子动量足够高时,电子可以在纵向上被捕获,这就是电子的纵向自注入。当激光光强较强并且自聚焦增强时,等离子体波由非线性正弦型向极端非线性即空泡结构演化,其背景电子会被横向离轴排开形成电子空腔。当背景电子的横向动量足够大时,电子可以进入等离子体电子空腔,在聚焦力的作用下进入加速区域,从而实现注入,即电子的横向自注入。横向注入会给电子束引入较大的发散角和发射度,而纵向注入会产生发射度较小的电子束。相比于其他注入方式,自注入对实验靶结构和等离子体密度分布的要求不高,但需要较高的激光强度来激发强等离子体波,而且自注入过程往往是不可控的。因此,在实验过程中,自注入在实现高品质的电子束注入方面具有一定的难度。
碰撞注入是利用另外一束激光脉冲激发电子额外注入的,也叫光学注入。1996年,Umstadter等[33]利用注入激光脉冲沿垂直方向作用于尾波场,该脉冲的有质动力能够加速等离子体中的一部分电子,使其能够被尾波场捕获。为了使电子注入到单个尾波场空泡中,注入脉冲的焦斑大小和脉冲宽度都要小于等离子体波长。此外,需要在时间上精准控制驱动尾波场的主激光脉冲和注入激光脉冲才能完成有效的电子注入。与横向对撞实现电子注入的方案有所不同,两束激光相向对撞的光学注入方案[34-35]来源于激光尾波场拍频注入的想法。在两束脉冲交叠的时间内,泵浦脉冲的尾部与对撞脉冲共振激发拍频等离子体波,从而尾波场会捕获和加速等离子体中的背景电子。由于碰撞注入在实验中要求超高精度的同步控制,且注入激光与等离子体的相互作用会对驱动激光造成影响,实现碰撞注入和持续的加速相对较难,稳定性也比较差。
电离诱导注入[36-39]则是基于激光与含有高Z元素(Z为物质的原子序数)的低密度气体之间的相互作用,高Z离子的内壳层电子直接在尾波场内被电离并被尾波场捕获和加速。当超强(强度>1018 W·cm-2)激光在等离子体中传播时,内壳层的电子由于电离势高,仅在激光脉冲峰值附近发生电离,而这些电子本身就处在空泡中,因此可以直接被捕获和加速。这种在空泡形成过程中尚未电离出来的电子的电离阈值要高于或者接近于激光脉冲的峰值功率,由于激光在空泡前端的自聚焦和自压缩,功率密度被提高到了电离阈值以上,直接在空泡中电离出新的电子。只要这些电子的初始位置合适,就能够在空泡内加速到足够快的速度,从而被空泡俘获实现注入。相比于自注入和碰撞注入,含有高Z元素的低密度气体的电离注入具有很强的优势,其对激光强度的要求相对较低,很容易实现。但电子的注入过程受限于激光在等离子体中的自聚焦传输,电离注入时间无法得到有效控制,往往会持续注入,最终导致输出的电子束电量较高、能散度较大。为了解决这个问题,研究者提出多种方案,如级联尾波场加速和自终止电离注入[40-41]方案。由
![激光尾波场的级联电子加速[40]。经离轴抛物面镜聚焦后,焦斑尺寸约为16 μm、峰值强度为2.08~2.27的驱动激光在(a)激光聚焦位置z=0且靶上激光功率为53 TW,(b)z=0.6 mm且靶上激光功率为58 TW,(c)z=1.2 mm且靶上激光功率为60 TW的打靶条件下,单独打第一级时得到的电子束能谱;在靶上激光功率分别为(d)48 TW和(e)60 TW的条件下,第二级等离子体长为1 mm时的级联电子加速电子能谱;在靶上激光功率分别为(f)50 TW、(g)48 TW和(h)45 TW的条件下,第二级等离子体长为3 mm时级联电子加速得到的电子束能谱](/richHtml/zgjg/2024/51/1/0101002/img_03.jpg)
图 3. 激光尾波场的级联电子加速[40]。经离轴抛物面镜聚焦后,焦斑 、峰值强度为2.08~2.27的驱动激光在(a)激光聚焦位置z=0且靶上激光功率为53 TW,(b)z=0.6 mm且靶上激光功率为58 TW,(c)z=1.2 mm且靶上激光功率为60 TW的打靶条件下,单独打第一级时得到的电子束能谱;在靶上激光功率分别为(d)48 TW和(e)60 TW的条件下,第二级等离子体长为1 mm时的级联电子加速电子能谱;在靶上激光功率分别为(f)50 TW、(g)48 TW和(h)45 TW的条件下,第二级等离子体长为3 mm时级联电子加速得到的电子束能谱
Fig. 3. Cascaded electron acceleration of laser wakefield[40]. After being focused by off-axis parabolic mirror, single-shot electron beam spectra from drive laser with focal spot size of ~16 μm and peak intensity of 2.08‒2.27 when (a) laser focusing position z=0 and laser power on target is 53 TW, (b) z=0.6 mm and laser power on target is 58 TW, and (c) z=1.2 mm and laser power on target is 60 TW; cascaded electron acceleration spectra when second stage plasma is 1 mm long at target powers of (d) 48 TW and (e) 60 TW; electron beam energy spectra obtained by cascaded electron acceleration when second stage plasma length is 3 mm at laser powers on target of (f) 50 TW, (g) 48 TW, and (h) 45 TW
在密度梯度注入的方法[42-43]中,激光在等离子体中传输时等离子体波会随着等离子体密度的变化,相速度相应发生变化,从而实现电子的局部自注入,其主要以下降沿的密度梯度注入为主。当脉冲激光沿密度下降沿(dn/dz<0,其中n为等离子体密度)传播时,等离子体波的周期会随着密度的减小而增大(λp∝n0-1/2),尾波场尾部的相速度会随着激光的传输逐渐减小。如在等离子体密度的下降区域n1→n2(n1>n2)内,等离子体波的本征波长增大,即λp1→λp2(λp1<λp2),该密度转换造成相峰值位置的变化量为∆ζ=λp1-λp2。如果密度下降沿长度为L,则等离子体尾波场的相速度变化量为∆vp/c=(λp1-λp2)/L=-dλp/dz=[λp/(2n)](dn/dz)。所以对于密度下降沿,尾波场的相速度是减小的,当尾波场尾部电子速度大于尾波场尾部相速度时,电子会被捕获。实验中制造等离子体密度下降沿的方法有很多,其中包括使用垂直于主脉冲的另一束脉冲激光、光预制等离子体通道和使用刀片制造气体冲击波,从而实现等离子体密度的陡峭变化等[44-47]。密度下降沿注入能够大大降低电子注入的能量阈值,从而降低驱动激光的强度,是一种非常有效的注入方案。值得指出的是,在不同的注入机制和加速过程中,如
3 高品质激光等离子体尾波场电子加速中的能量啁啾控制
激光等离子体尾波场电子加速主要包括四个过程:激光尾波场的形成和演化、电子注入、电子加速以及失相过程[5,67]。在电子加速阶段,电子被注入到尾波场后会受到纵向尾波场的加速而能量增加。当电子速度增加到超过尾波场相速度时,电子束会相对于尾波场由尾部向中间移动,发生相对滑移。当加速长度(Ld)等于失相长度时,即Ld∝λ
为得到高性能低能散度的电子束团,国际小组针对激光尾波场电子加速的注入和加速过程提出了各种各样的优化方案。2006年,Faure等[53]采用两束超短脉冲激光相向对撞实现电子束注入的方案,在实验上获得了能量为117 MeV、半峰全宽(FWHM)能散度为11%的电子束团输出。2015年,Mirzaie等[58]在不匹配的焦斑条件下,加剧了激光自聚焦的演化,从而抑制了电子束的电离注入过程,在实验上得到了能量为412 MeV、半峰全宽能散度为5%的电子束团输出。2013年,Buck等[44]利用气体激波产生的密度陡梯度下降沿,对电子束的注入过程进行控制,在实验中获得了能量为~100 MeV、半峰全宽绝对能散度为~5 MeV的稳定电子束团输出。2014年,Litos等[72]针对电子束驱动等离子体加速器设计的尾波场平坦化方案可控制被加速的电子束团保持稳定的绝对能散度,从而抑制电子束团的能散度增长。但对于激光驱动的尾波场来说,电子束存在失相效应,电子束团并不能保持稳定的加速相位,而且电子束的能量啁啾没有得到有效的补偿或者控制,因而上述激光尾波场加速方案产生的电子束能散度都在百分之几的量级,与诸多应用所要求的千分之一级能散度还有很大的差距。
为了得到能散度更低的高能电子束,需要对电子束团在尾波场内的加速过程进行精确控制。对于在激光尾波场加速初始阶段注入的电子束团来说,先被捕获的电子的加速时间长,会获得更高的能量增益,因此形成能量呈负啁啾分布(电子束团能量分布前高后低)的电子束团。由于尾波场的加速梯度在纵向上不是均匀分布的,而是存在两个区域,一个是加速梯度负斜率区,另一个是加速梯度正斜率区,电子束在被加速的同时,其能量啁啾会发生演化,经历啁啾补偿(去啁啾)和翻转的过程。因此,通过控制加速过程中能量啁啾的演化可以减小或者压缩电子束的绝对能散度,从而获得低能散度的高能电子束。研究者针对传统加速器领域也提出了各种去啁啾方案[73-77],如对电子进行能量啁啾度操控,就是利用一个合适的场与带有能量啁啾的电子束的相互作用来消除电子束的能量啁啾度。这种方法在基于激光尾波场的电子加速中也是适用的。如2016年,Zhang等[78]提出了在相空间中进行电子束纵向压缩、电子束能量啁啾翻转和压缩电子束能散度补偿的新方案,这为激光尾波场加速产生千分之一级低能散度、高流强、高亮度电子束提供了新思路。2016年,Wang等[18]利用具有特殊密度分布的级联激光尾波场加速器有效地抑制了电子的二次自注入,控制了电子束在加速过程中的能量啁啾演化,在实验中获得了峰值能量为200∼600 MeV、能散度为0.4%~1.2%、电量为10~80 pC、发散角为0.1~0.3 mrad的高品质单能电子束。该电子束最高的六维相空间亮度达到6.5×1015 A·m-2/0.1%(0.1%为均方根能散度单位),可比拟世界上最先进的传统直线加速器产生的高亮度高品质电子束团。2021年,Ke等[65]通过研究具有陡峭下降沿的等离子体密度分布,提出通过控制电子束注入、去啁啾和准稳相加速获得超低能散度的高亮度、高能电子束的方案,最终在实验上获得了能量为780~840 MeV、能散度的均方根值(RMS)为2.4‰~4.1‰、电量为8.5~23.6 pC、发散角的RMS为0.1~0.4 mrad的高品质电子束团,并通过模拟进行了验证。通过模拟发现,该电子束团的啁啾强度高达11 TeV·mm-1·m-1,并且通过调节等离子密度或者激光焦点位置等,可以有效调节电子束的初始啁啾度以及去啁啾强度,进而获得无啁啾的电子束。该方法适用于获取相对能散度在0.5%以下的电子束。
高品质激光尾波场电子加速实验示意图如
![基于激光尾波场加速的超低能散、高品质电子束源产生实验[65]。(a)高品质激光尾波场电子加速实验示意图;(b)阴影法观测到的激波分布;(c)沿着图4(b)虚线方向的干涉图反演得到的密度分布(实线),真空中激光的演化(虚线),及等离子体中激光的演化(阴影区域)](/richHtml/zgjg/2024/51/1/0101002/img_04.jpg)
图 4. 基于激光尾波场加速的超低能散、高品质电子束源产生实验[65]。(a)高品质激光尾波场电子加速实验示意图;(b)阴影法观测到的激波分布;(c)沿着图4(b)虚线方向的干涉图反演得到的密度分布(实线),真空中激光的演化(虚线),及等离子体中激光的演化(阴影区域)
Fig. 4. Experiments for generation of ultralow energy-spread and high-quality electron beam based on laser-wakefield acceleration[65]. (a) Schematic of experimental setup for high-quality laser wakefield electron acceleration; (b) shock wave distribution observed by shadow method; (c) retrieved plasma density profile (solid line) from interferogram along dashed line direction in Fig.4(b) and evolution of laser beam in vacuum (dashed line) and in plasma (shaded area)
![超低能散、高品质电子束源的实验测量结果[65]。(a)实验测得的能散为2.4‰~4.1‰的15发电子能谱;(b)图5(a)中第13发电子束的电量积分图,其峰值能量为817 MeV,RMS能散度为3.3‰,阴影部分表示三倍相对能散范围内的电子束电量为10.6 pC,RMS发散角为0.25 mrad](/richHtml/zgjg/2024/51/1/0101002/img_05.jpg)
图 5. 超低能散、高品质电子束源的实验测量结果[65]。(a)实验测得的能散为2.4‰~4.1‰的15发电子能谱;(b)图5(a)中第13发电子束的电量积分图,其峰值能量为817 MeV,RMS能散度为3.3‰,阴影部分表示三倍相对能散范围内的电子束电量为10.6 pC,RMS发散角为0.25 mrad
Fig. 5. Experimental measurement results for ultralow energy-spread and high-quality electron beam source[65]. (a) Energy spectra of 15 shots with energy spread of 2.4‰‒4.1‰ measured by experiment; (b) charge integral diagram of 13th shot electron beam with peak energy of 817 MeV and RMS energy spread of 3.3‰ in Fig.5(a), with electron beam charge of 10.6 pC in threefold relative energy spread range and RMS divergence angle of 0.25 mrad shown in shaded portion
在模拟实验中采用了傅里叶贝塞尔粒子模拟程序(FBPIC)[82-83]。模拟窗口的纵向以及横向尺寸分别为50 μm和120 μm,其中每个格点包含16个宏粒子,尺寸为Δz=0.4 μm,Δr=0.12 μm。一束波长为0.8 μm、归一化强度为1.24、脉宽为25 fs、焦斑直径为34 μm的线偏振激光从模拟窗口的左边纵向入射并聚焦于距离等离子体出口1.4 mm的位置。在模拟实验中,激光脉冲用高斯函数表示。等离子体密度分布非常接近实验测量的结果,如
![粒子网格模拟结果[65]。(a)归一化矢势、尾波场相速度以及等离子密度的演化图,归一化矢势的演化可以粗略地分成振荡阶段(中间阴影区域)以及近匹配阶段(右边阴影区域);(b)电子束能量和绝对能散度的演化;(c)电子束拟合的啁啾度以及所在空间位置的平均纵向场](/richHtml/zgjg/2024/51/1/0101002/img_06.jpg)
图 6. 粒子网格模拟结果[65]。(a)归一化矢势、尾波场相速度以及等离子密度的演化图,归一化矢势的演化可以粗略地分成振荡阶段(中间阴影区域)以及近匹配阶段(右边阴影区域);(b)电子束能量和绝对能散度的演化;(c)电子束拟合的啁啾度以及所在空间位置的平均纵向场
Fig. 6. Particle-in-cell simulation results[65]. (a) Evolution diagram of normalized vector potential, wakefield phase velocity, and plasma density, in which evolution of normalized vector potential can be roughly divided into oscillation stage (middle shadow region) and near-matching stage (right shadow region); (b) evolution of electron beam energy and absolute energy spread; (c) chirp degree fitted by electron beam and mean longitudinal field at its spatial location
当密度下降沿结束时,随着尾波场相速度的增大,电子注入也结束了。电子刚注入空泡时,电子束头部的电子先注入,比尾部的电子获得了更多的能量,有了负的能量啁啾度。此时的加速场梯度的斜率是正的,尾部的电子比头部的电子承受更高的加速梯度,如
![电子束在roz平面上的密度分布、光轴上的尾场分布(实线),以及不同传输位置相空间运动情况[65]。(a)电子束注入后处在一个高度非线性的尾波场中;(b)当激光散焦时,形成了一个有负斜率加速梯度的锯齿状加速场;(c)beam loading效应使得电子在后面的加速过程都保持比较小的相对能散度;(d)电子束接近失相;(e)~(h)对应于图7(a)~(d)的相空间动力学以及局部放大纵向尾波场](/richHtml/zgjg/2024/51/1/0101002/img_07.jpg)
图 7. 电子束在roz平面上的密度分布、光轴上的尾场分布(实线),以及不同传输位置相空间运动情况[65]。(a)电子束注入后处在一个高度非线性的尾波场中;(b)当激光散焦时,形成了一个有负斜率加速梯度的锯齿状加速场;(c)beam loading效应使得电子在后面的加速过程都保持比较小的相对能散度;(d)电子束接近失相;(e)~(h)对应于图7(a)~(d)的相空间动力学以及局部放大纵向尾波场
Fig. 7. Snapshots of electron beam density distribution in roz plane, on-axis accelerating field lineouts (solid line), and phase space dynamics of electron beam at different distances. (a) Electron beam stays in a highly nonlinear wakefield just after injection; (b) sawtooth-like accelerating field with negative slope acceleration gradient is formed as laser defocuses; (c) beam-loading effect makes electrons maintain relatively small energy spread during final acceleration process; (d) electron beam approaches dephasing; (e)‒(h) corresponding phase space dynamics and locally amplified longitudinal wakefield for Fig.7(a)‒(d)
4 激光驱动的betatron X射线辐射
当相对论运动的电子受到外力发生偏转或者切向加速时,能够对外辐射高能量光子,其电子辐射的特性,如角分布、辐射能谱、光子产额、偏振态以及时间空间相干性等,与电子束参数和运动状态紧密相关。激光尾波场加速的电子束可以在等离子体、光场、强磁场中进行横向振荡或扭摆运动产生高能量、高亮度、准直、飞秒脉宽的新型辐射源[13],如betatron X射线辐射、逆康普顿散射、韧致辐射和波荡器辐射等,可覆盖从极紫外到伽马射线的极宽能带光谱。除此之外,Malaca等[85]提出利用等离子体加速器产生具有时间相干的超辐射,Peng等[86]提出在超光速等离子体尾波场中采用基于电子集体作用的全新相干辐射机制,该机制可产生阿秒量级脉冲、亚周期相干光激波辐射。
在激光尾波场加速电子过程中,注入到空泡内的电子除了在纵向上受到一个100 GV/m量级的超高加速场外,在激光尾波场的横向聚焦场作用下,电子也会在横向上作简谐振动,从而产生betatron辐射,如
式中:
![电子在空泡中的betatron振荡和betatron辐射[87]。(a)betatron振荡;(b)betatron辐射](/richHtml/zgjg/2024/51/1/0101002/img_08.jpg)
图 8. 电子在空泡中的betatron振荡和betatron辐射[87]。(a)betatron振荡;(b)betatron辐射
Fig. 8. Betatron oscillation and radiation of electron in bubble[87]. (a) Betatron oscillation; (b) betatron radiation
可以通过增加电子束的电量和能量或增大电子束的横向振荡振幅来提高betatron辐射的光子能量和产额。以往的研究报道了几种提高betatron辐射光子能量和产额的方法。2011年,Cipiccia等[19]利用激光尾波场内电子束与驱动激光产生的共振增强横向振荡,获得了MeV量级的辐射源。2008年,Chen等[20]和Yan等[88]利用团簇喷嘴气池和等离子体空泡的演化,提高了辐射源的光子产额和能量。2018年,Ferri等[89]采用激光尾波场电子加速和电子束驱动的尾波场混合协同作用的方法,产生了高亮度MeV量级的betatron辐射。2019年,Guo等[90]利用密度抑制等离子体结构,实现了激光尾波场中的betatron辐射增强。2020年,Zhu等[91]利用几拍瓦量级的强激光在级联激光等离子体加速中获得了超高亮度GeV级的伽马射线源。此外,2010年,Popp等[92]利用波前倾斜的激光引导离轴电子的注入,实现了对辐射源的操控。2016年,Chen等[21]提出了利用厘米尺寸大小的等离子体通道产生类同步辐射源的方案。2018年,Döpp等[93]还实现了该辐射源的偏转态调谐控制等。2023年,Shou等[94]利用激光加速的电子在毫米长度碳纳米管内形成的近临界密度等离子体,通过类同步辐射获得了高光子产额的硬X射线,其转换效率高达10-3。但由于这些电子束的能散度并不好,是连续分布的,且可控性也不够,所产生的X射线辐射往往为连续宽能谱分布,缺乏一定的可控性。因此需要进一步探索能产生稳定、易于调谐、准单色betatron辐射源的方法,从而提高betatron射线源的应用性能。
然而,激光尾波场内的电子束注入和加速过程是紧密相连的,若要产生低能散度、低发射度、高电量的电子束,则要求注入电子束的横向振荡不能太大,否则在加速过程中电子束的品质难以维持,从而限制betatron辐射的光子产额。此外,由于电子束在尾波场中难以维持稳定的电子能量,加速过程中电子束横向振幅的变化以及激光尾波场结构的自身演化会大大限制辐射光子的单能性。如果电子束的注入、加速和横向振荡可以被独立控制,则很有可能解决高品质电子束和增强betatron辐射之间的矛盾,同时优化电子束产生和betatron辐射,获得一种紧凑型、高亮度、高能量的电子束源及经过增强的betatron辐射源。
2018年,Yu等[22]提出了一种在激光尾波场内操控电子束横向振荡的新方案,通过激光级联尾波场机制将电子束的注入和不同空泡内的级联加速过程分开,在加速阶段巧妙地引入倾斜的等离子体折射平板,驱动激光经过这一特殊结构发生折射,而加速的准单能电子束的传播方向不变,激光与电子束之间的不同轴传输可以极大增加电子束的横向动量,使电子束在尾波场中的横向振荡振幅增大[22]。如
![在激光尾波场内利用倾斜等离子体折射平板操控电子束及betatron辐射的示意图[22]](/richHtml/zgjg/2024/51/1/0101002/img_09.jpg)
图 9. 在激光尾波场内利用倾斜等离子体折射平板操控电子束及betatron辐射的示意图[22]
Fig. 9. Schematic of electron beam and betatron radiation manipulated by tilted plasma refraction slab in laser wakefield[22]
当激光从左向右传播时,在三段不同密度的等离子体中其折射率不同,折射率表达式为η=[1-
在实验中,为产生倾斜的等离子体密度分布,如
![激光尾波场电子加速及增强betatron辐射实验[22]。(a)实验装置示意图;(b)利用光学干涉测量法及阴影法测量的倾斜等离子体平板密度分布](/richHtml/zgjg/2024/51/1/0101002/img_10.jpg)
图 10. 激光尾波场电子加速及增强betatron辐射实验[22]。(a)实验装置示意图;(b)利用光学干涉测量法及阴影法测量的倾斜等离子体平板密度分布
Fig. 10. Experiments of laser wakfield electron acceleration and enhanced betatron radiation[22]. (a) Schematic of experimental setup; (b) density distribution of measured tilted plasma slab via optical interferometry and shadowgraphy
![实验测量结果[22]。(a)~(d)引入倾斜等离子体激波前后在不同位置测得的单发电子能谱;(e)引入倾斜等离子体激波前后电子束束斑的中心位置分布情况;(f)当倾斜等离子体激波在不同位置时,电子束的平均电量和betatron辐射源的峰值能量及总光子产额](/richHtml/zgjg/2024/51/1/0101002/img_11.jpg)
图 11. 实验测量结果[22]。(a)~(d)引入倾斜等离子体激波前后在不同位置测得的单发电子能谱;(e)引入倾斜等离子体激波前后电子束束斑的中心位置分布情况;(f)当倾斜等离子体激波在不同位置时,电子束的平均电量和betatron辐射源的峰值能量及总光子产额
Fig. 11. Experimental measurement results[22]. (a)‒(d) Single-shot electron energy spectra measured at different positions before and after introducing tilted plasma shock; (e) distribution of central position of electron beam spot before and after introducing tilted plasma shock ; (f) measured average charge of electron beam and peak energy and total photon yield of betatron radiant source when tilted plasma shock wave is at different positions
实验中采用单光子模式和衰减片两种方案测量betatron辐射的能谱。如
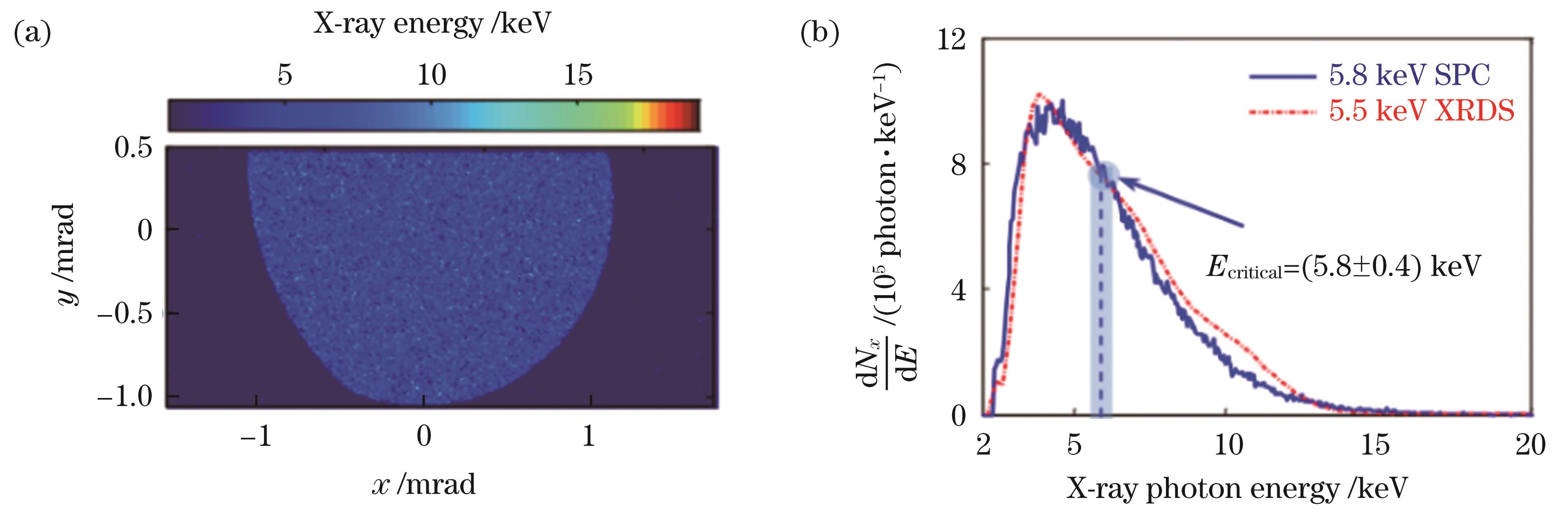
图 12. 没有倾斜等离子体平板时的betatron辐射诊断。(a)在X射线电荷耦合器件(CCD)上的典型betatron辐射的光子分布;(b)基于单光子(SPC)模式和X射线测量系统(XRDS)测得的betatron辐射能谱
Fig. 12. Diagnosis of betatron radiation without tilted plasma slab. (a) Typical betatron radiation photon distribution recorded on X-ray charge coupled device (CCD); (b) measured spectra of betatron radiation based on single-photon-counting (SPC) mode and X-ray detection system (XRDS)
相比之下,当引入倾斜等离子体平板增强betatron辐射时,单光子模式测量方法将不再适用,需要采用衰减片阵列的X射线测量系统进行能谱测量[22,24]。通过实验优化,测得增强后的betatron辐射源空间分布如
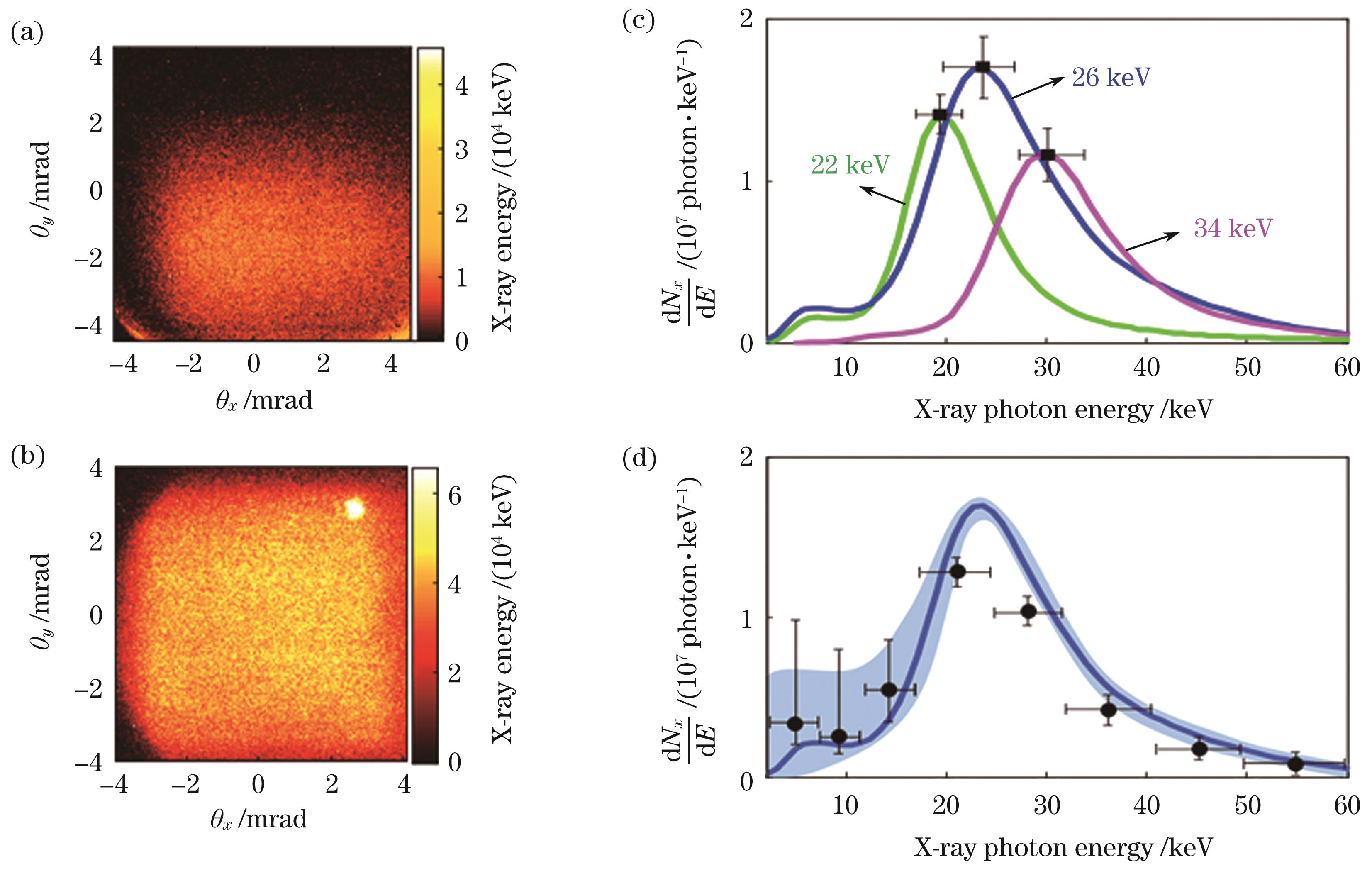
图 13. 增强betatron辐射实验结果。(a)没有折射板和(b)有折射板增强betatron辐射情况下的X射线源空间分布;(c)倾斜等离子体平板在不同位置的辐射能谱;(d)重构的增强betatron辐射源能谱(实线)
Fig. 13. Experimental results of enhanced betatron radiation. Spatial distributions of X-ray sources (a) without and (b) with refractor enhanced betatron radiation; (c) radiation spectra of tilted plasma slab at different locations; (d) retrieved spectra of enhanced betatron radiation (solid line)
PIC模拟展示了利用倾斜板操控高品质电子束产生和增强betatron辐射的机制和过程,如
![粒子模拟结果[22]。(a)引入倾斜等离子体平板的等离子体密度分布;在(b)有倾斜等离子体平板和(c)没有倾斜等离子体平板的情况下,注入电子束和尾波场结构的演化,右侧为在两种不同情况下激光传播9.2 ps后的电子能谱分布;(d)在有和没有倾斜等离子体平板时,电子束的横向尺寸、电子束横向振荡和部分电子轨迹追踪;(e)当倾斜等离子体平板在不同位置时,电子束峰值能量和RMS能散度的演化,黑线为没有倾斜等离子体平板时的模拟结果](/richHtml/zgjg/2024/51/1/0101002/img_14.jpg)
图 14. 粒子模拟结果[22]。(a)引入倾斜等离子体平板的等离子体密度分布;在(b)有倾斜等离子体平板和(c)没有倾斜等离子体平板的情况下,注入电子束和尾波场结构的演化,右侧为在两种不同情况下激光传播9.2 ps后的电子能谱分布;(d)在有和没有倾斜等离子体平板时,电子束的横向尺寸、电子束横向振荡和部分电子轨迹追踪;(e)当倾斜等离子体平板在不同位置时,电子束峰值能量和RMS能散度的演化,黑线为没有倾斜等离子体平板时的模拟结果
Fig. 14. Particle-in-cell simulation results[22]. (a) Plasma density distribution with tilted plasma slab; evolution of injected electron beam and wakefield structures (b) before and (c) after introducing tilted plasma slab with corresponding electron energy spectra after 9.2 ps laser propagation shown on right; (d) transverse size and transverse oscillation of electron beam and partial electron trajectory tracking without and with tilted plasma slab; (e) evolutions of peak energy and RMS energy spread of electron beams when tilted plasma slab is placed at different positions with simulation result without tilted plasma slab shown by black curve
5 激光驱动的逆康普顿散射
当相对论电子与激光发生对撞时,可以通过逆康普顿散射过程实现光子能量上转换并产生高能光子。假设与电子束对撞的激光为线偏振光,考虑对撞激光的中心波长为800 nm,则对撞所辐射出的光子能量为
式中:
基于激光尾波场加速的相对论电子束与激光对撞的逆康普顿散射是目前产生台式化高能伽马射线源的最有效途径之一。2006年,Schwoerer等[98]将一束能量为370 mJ的飞秒激光分成两束,一束能量为330 mJ,驱动尾波场产生MeV量级电子束,与另一束激光对撞首次产生了基于激光尾场电子加速的全光汤姆森散射源。2013年,Chen等[99]通过提高电子束能量和对撞激光强度将射线能量提高到4 MeV,如
![利用两束激光驱动全光逆康普顿散射产生4 MeV伽马射线的实验示意图[99]](/richHtml/zgjg/2024/51/1/0101002/img_15.jpg)
图 15. 利用两束激光驱动全光逆康普顿散射产生4 MeV伽马射线的实验示意图[99]
Fig. 15. Experimental diagram of 4 MeV γ-ray generation based on all-optical inverse Compton scattering by two laser pulses[99]
为了避免复杂的准直和同步问题,2012年,Phuoc等[23]利用等离子体镜反射驱动激光与紧随其后的电子束对撞,如
![基于等离子体镜的全光逆康普顿背散射X射线源实验原理[23]](/richHtml/zgjg/2024/51/1/0101002/img_16.jpg)
图 16. 基于等离子体镜的全光逆康普顿背散射X射线源实验原理[23]
Fig. 16. Experimental principle of all-optical inverse Compton backscattering X-ray source based on plasma mirror[23]
实验装置示意图如
![全光自同步康普顿散射实验装置图[24]](/richHtml/zgjg/2024/51/1/0101002/img_17.jpg)
图 17. 全光自同步康普顿散射实验装置图[24]
Fig. 17. Experimental setup for all-optical self-synchronizing Compton scattering[24]
实验结果表明,所产生的逆康普顿射线源在水平和垂直方向上的半峰全宽发散角分别为3.8 mrad和4.1 mrad,如
![激光尾波场电子加速驱动的全光逆康普散射实验结果[24]。(a)扣除背景后具有高斯分布的伽马射线束斑;(b)经过不同衰减片后的束斑分布;(c)薄膜在z=10 mm处时的背景辐射;(d)~(f)被探测系统上相应的光子分布;(g)伽马射线源及背景在横向和纵向方向上的强度分布;(h)不同能量电子束产生的伽马射线源能谱](/richHtml/zgjg/2024/51/1/0101002/img_18.jpg)
图 18. 激光尾波场电子加速驱动的全光逆康普散射实验结果[24]。(a)扣除背景后具有高斯分布的伽马射线束斑;(b)经过不同衰减片后的束斑分布;(c)薄膜在z=10 mm处时的背景辐射;(d)~(f)被探测系统上相应的光子分布;(g)伽马射线源及背景在横向和纵向方向上的强度分布;(h)不同能量电子束产生的伽马射线源能谱
Fig. 18. Experimental results of all-optical inverse Compton scattering driven by laser wakefield electron acceleration[24]. (a) Typical background subtracted Gaussian-profile γ-ray beam spot; (b) beam spot distribution after passing different attenuating plates; (c) background radiation when film is placed at z=10 mm; (d)‒(f) corresponding photon distribution on detected system; (g) horizontal and vertical intensity distributions of γ-ray source and background; (h) γ-ray source spectra produced by electron beams with different energies
式中:
实验中,所产生的伽马射线能谱宽度主要来源于电子束能散度、发射度、激光带宽、激光强度以及探测角度的共同作用。激光在等离子体中的传输会引起光子加速、离化和局部泵浦损耗,从而引起反射激光的蓝移和红移。反射激光的红移和蓝移会展宽伽马射线能谱,且伽马光子会红移到更低的能量。另外,电子束每发之间的指向性抖动也会引起探测角的偏离(<1.2 mrad),进一步展宽测得的伽马射线能谱。以伽马射线峰值能量为0.53 MeV、半峰全宽为~33%的测量结果为例,电子束的能散度(~3%)和发射度(~1.2 mm·mrad)引起的射线能谱展宽只占~8%。而增加的能谱展宽则来源于伽马射线能谱重建精度(~15%),以及反射激光谱宽、离轴探测、反射激光的有质动力(~25%)的卷积作用。
需要指出的是,薄膜的相对位置在逆康普顿散射过程中有着重要的作用,为了提高伽马射线的光子产额,需要根据实际情况进行调谐和优化。当实验采用不同的加速长度时,激光尾波场加速的电子束能量从160 MeV增加到420 MeV,对应的伽马射线峰值能量也从0.3 MeV提高到2.0 MeV,如
![全光逆康普顿散射产生可调谐的伽马射线源[24]。(a)不同电子束峰值能量下测得的伽马射线峰值能量,虚线为拟合曲线,横向和纵向误差棒分别代表电子束峰值能量和伽马射线峰值能量的不确定度;(b)伽马射线光子数与薄膜位置之间的关系,实线为薄膜在z=6 mm处的韧致辐射背景平均值;(c)九方格滤光器在z=0 mm和z=5 mm处的伽马射线束斑图样](/richHtml/zgjg/2024/51/1/0101002/img_19.jpg)
图 19. 全光逆康普顿散射产生可调谐的伽马射线源[24]。(a)不同电子束峰值能量下测得的伽马射线峰值能量,虚线为拟合曲线,横向和纵向误差棒分别代表电子束峰值能量和伽马射线峰值能量的不确定度;(b)伽马射线光子数与薄膜位置之间的关系,实线为薄膜在z=6 mm处的韧致辐射背景平均值;(c)九方格滤光器在z=0 mm和z=5 mm处的伽马射线束斑图样
Fig. 19. Tunable γ‑ray sources generated from all-optical inverse Compton scattering[24]. (a) Measured γ‑ray peak energy versus electron beam peak energy with fitted curve shown by dashed line, and uncertainties of electron beam and γ-ray peak energies shown by horizontal and vertical error bars; (b) relationship between number of γ-ray photons and position of film with mean bremsstrahlung background of film at z=6 mm shown by solid line; (c) γ-ray beam spot patterns of nine-grid filter at z=0 mm and z=5 mm
每次打靶后需要左右移动薄膜的位置(保持z不变),从而保证每次激光与薄膜相互作用时薄膜表面的完整性。如
全光逆康普顿散射源的大小与电子束参数近似,结合三维PIC模拟结果,激光驱动的逆康普顿散射源的横向尺寸和脉宽分别为~4 μm和~6 fs,根据实验测得的伽马射线光子数(~5×107/shot)、能谱分布和束斑发散角,可大体估算出产生的MeV量级伽马射线源的峰值亮度高达(3.1±0.4)×1022 photon·s-1·mm-2·mrad-2@0.1%(0.1%为带宽)。这种伽马射线源亮度的提高主要得益于激光级联尾波场加速产生的低能散度、低发射度、高电量的高品质电子束,以及这种自同步逆康普顿对撞机制。通过进一步提高激光打靶频率以及电子束电量等,也可提高伽马射线源的平均亮度,从而提升其应用性能。
6 基于激光尾波场加速的台式化自由电子激光
自由电子激光被称为第四代光源,可以提供从远红外到X射线波段的高亮度相干辐射,在物理、化学、材料科学、生命科学等领域中具有前所未有的应用价值。X射线自由电子激光(X-FEL)一直是世界各国科学家关注和研究的热点,但现有的传统X-FEL装置,如上海光源[107]、LCLS、SACLA、DESY、FERMI等,往往存在光源结构复杂、占地大、花费高等缺点。激光尾波场电子加速器近些年来得到了长足发展,在发展新型飞秒相干X射线源方面有广阔的应用前景和巨大的应用潜力,有望实现紧凑型台式化的X射线自由电子激光器。
平面正弦静磁场波荡器的强度为K=eB0/(mecku)=0.94B0λu(
式中:k为辐射场波势;θ为束团的有质动力势相位;
高品质电子束可在波荡器磁场中作正弦振荡,与辐射的光场发生耦合作用,放大辐射出高强度、高亮度、横向相干、窄谱宽、波长可调谐的光子。这种自放大自发辐射模式是当今主流的高增益自由电子激光工作模式,由于不需要谐振腔或外界的种子源,理论上可以工作于任意波段。自由电子激光的工作波长满足的共振条件为
自放大自发辐射过程主要包括自发辐射、指数增益和饱和三个阶段。
![激光尾波场加速的电子束通过传统波荡器产生的同步辐射示意图[13,108]。(a)同步辐射;(b)自放大自发辐射(SASE)](/richHtml/zgjg/2024/51/1/0101002/img_20.jpg)
图 20. 激光尾波场加速的电子束通过传统波荡器产生的同步辐射示意图[13,108]。(a)同步辐射;(b)自放大自发辐射(SASE)
Fig. 20. Schematics of synchrotron radiation generated by laser-wakefield accelerated electron beam passing through conventional undulators[13,108]. (a) Synchrotron radiation; (b) self-amplified spontaneous emission (SASE)
![激光尾场加速电子束通过波荡器后产生软X射线辐射的实验装置图[109]](/richHtml/zgjg/2024/51/1/0101002/img_21.jpg)
图 21. 激光尾场加速电子束通过波荡器后产生软X射线辐射的实验装置图[109]
Fig. 21. Experimental setup of soft X-ray radiation after laser-wakefield accelerated electron beam passing through undulator[109]
近10年来,激光驱动的尾波场加速在高品质高能电子束的产生,特别是在能散度的压缩和窄化方面取得了重要进展,实验获得了3‰能散度的GeV级高能电子束[18,65],为实现小型化的自由电子激光器奠定了基础。基于该电子束,2021年,Wang等[110]采用4.5 m长的传统波荡器获得了极紫外波段的自发辐射放大输出,实现了台式化自由电子激光原理的实验验证。实验装置示意图如
![基于激光尾波场加速的紧凑型自由电子激光辐射装置示意图和相关数据[110]。(a)波荡器束线;(b)典型电子束能谱;在波荡器(c)入口处和(d)出口处测得的电子束横向分布](/richHtml/zgjg/2024/51/1/0101002/img_22.jpg)
图 22. 基于激光尾波场加速的紧凑型自由电子激光辐射装置示意图和相关数据[110]。(a)波荡器束线;(b)典型电子束能谱;在波荡器(c)入口处和(d)出口处测得的电子束横向分布
Fig. 22. Schematic of compact free-electron-laser radiation device based on laser wakefield acceleration and relate data[110]. (a) Undulator beamline; (b) typical spectrum of electron beam; measured transverse profiles of electron beam at (c) entrance and (d) exit of undulator
通过提升激光尾波场电子束的品质与稳定性,控制电子束的相空间演化,实现了电子束从等离子体到真空的平稳过渡,以及电子束的长距离传输,并将其有效耦合至波荡器中。如
![基于激光尾波场加速的自由电子激光辐射的实验结果[110]。(a)利用orbit kick方法测量得到的辐射能量(三角形)以及不用orbit kick方法测量得到的辐射能量(方块);(b)(c)对应的辐射分布图;(d)中心波长为27 nm的波荡器辐射能谱图及(e)对应的电子束能谱;(f)270发次的辐射能量](/richHtml/zgjg/2024/51/1/0101002/img_23.jpg)
图 23. 基于激光尾波场加速的自由电子激光辐射的实验结果[110]。(a)利用orbit kick方法测量得到的辐射能量(三角形)以及不用orbit kick方法测量得到的辐射能量(方块);(b)(c)对应的辐射分布图;(d)中心波长为27 nm的波荡器辐射能谱图及(e)对应的电子束能谱;(f)270发次的辐射能量
Fig. 23. Experimental results for free-electron-laser radiation based on laser wakefield acceleration[110]. (a) Measured radiation energy with (triangle) and without (square) orbit kick method; (b)(c) corresponding radiation patterns; (d) measured radiation spectra of undulator with central wavelength of 27 nm and (e) corresponding electron-beam energy spectra; (f) radiation energy of 270 shots
2022年,Pompili等[111]验证了传统光阴极电子源结合电子束驱动的等离子体尾波场加速(PWFA)在830 nm波段产生了自由电子激光。Labat等[112]利用激光尾场电子驱动的Seeded-FEL方案实现了辐射波长可控且具有高纵向相干的自由电子激光输出。此外,Habib等[113]提出了利用PWFA产生超低发射度、高亮度的阿秒级电子束,并将其耦合到后续的波荡器中,实现自由电子激光输出的理论方案。尽管激光驱动的高品质电子束以及自由电子激光研究在近年来取得了显著的进展,但与传统电子加速器产生的电子束和自由电子激光相比,电子束的品质特别是在稳定性、重复频率和转换效率等方面还有较大的差距,还需要优化相关技术以缩小差距。
7 总结和展望
相比于传统的射频电子加速器,飞秒强激光驱动的尾波场电子加速因具有加速梯度高、脉冲时间短以及与激光天然的高精度同步性能而备受青睐。飞秒强激光驱动的新型辐射及粒子束源,由于能量覆盖范围大,时间宽度短,在高能物理、材料科学、凝聚态物理、生命科学、医学及超快化学分子动力学等前沿交叉科学中具有广阔的应用前景[9-13,26,29,114]。因此,激光尾波场电子加速及其应用一直是强场激光物理和新型加速器领域的热点和重要研究方向。经过近20年的快速发展,激光尾波场电子加速实验和相关理论研究均取得了长足的进步,但是尚有很多关键的科学问题和技术难点需要解决,特别是激光驱动等离子体尾波场电子加速正处于从激光加速向激光加速器跨越的重要关口。虽然近10年来激光尾波场加速的电子束品质如能散度、发射度和稳定性等都得到极大的提高,但是其电子束的能散度、稳定性和重复频率与传统加速器加速的电子束相比还有较大的差距,特别是在综合性能指标上,尚不能达到应用要求。在获得低能散度、低发射度电子束的情况下,电子束的能量稳定性往往较差,重复频率较低,通常在1 Hz以下;而在电子束稳定性好的条件下,电子束的能散度和发散角又很大,这使得激光尾波场电子加速的应用受到很大的限制。造成这种困境的主要原因是所采用的电子注入和加速方案的可靠性以及激光器和气体喷流的稳定性有一定的缺陷。因此,如何在实验中更稳定、更可控地获得性能参数更优的电子束,如超高能量(10 GeV量级)、超低能散度(0.1%~0.01%)、超小发射度(~1 μm·mrad)、高重复频率、高稳定性等,是需要解决的问题。此外,也需要进一步探索提高新型辐射源的亮度和应用性能的方法。所幸随着超短脉冲激光技术的飞速发展,飞秒激光的脉宽越来越短,小型化的飞秒激光器的重复频率越来越高,稳定性也越来越可靠,激光波形也可以被精密操控,这将推动高品质激光尾波场电子加速器的持续发展。
[1] Strickland D, Mourou G. Compression of amplified chirped optical pulses[J]. Optics Communications, 1985, 56(3): 219-221.
[2] Mourou G, Tajima T. More intense, shorter pulses[J]. Science, 2011, 331(6013): 41-42.
[3] Krausz F, Brabec T, Schnürer M, et al. Extreme nonlinear optics: exposing matter to a few periods of light[J]. Optics and Photonics News, 1998, 9(7): 46-51.
[4] Tajima T, Dawson J M. Laser electron accelerator[J]. Physical Review Letters, 1979, 43(4): 267-270.
[5] Esarey E, Schroeder C B, Leemans W P. Physics of laser-driven plasma-based electron accelerators[J]. Reviews of Modern Physics, 2009, 81(3): 1229-1285.
[6] Pukhov A. Three-dimensional simulations of ion acceleration from a foil irradiated by a short-pulse laser[J]. Physical Review Letters, 2001, 86(16): 3562-3565.
[7] Esirkepov T, Borghesi M, Bulanov S V, et al. Highly efficient relativistic-ion generation in the laser-piston regime[J]. Physical Review Letters, 2004, 92(17): 175003.
[8] Yan X Q, Lin C, Sheng Z M, et al. Generating high-current monoenergetic proton beams by a circularly polarized laser pulse in the phase-stable acceleration regime[J]. Physical Review Letters, 2008, 100(13): 135003.
[9] Krausz F, Ivanov M. Attosecond physics[J]. Reviews of Modern Physics, 2009, 81(1): 163-234.
[10] Pukhov A. X-rays in a flash[J]. Nature Physics, 2006, 2(7): 439-440.
[11] Dromey B, Zepf M, Gopal A, et al. High harmonic generation in the relativistic limit[J]. Nature Physics, 2006, 2(7): 456-459.
[12] Gordienko S, Pukhov A, Shorokhov O, et al. Relativistic Doppler effect: universal spectra and zeptosecond pulses[J]. Physical Review Letters, 2004, 93(11): 115002.
[13] Corde S, Ta Phuoc K, Lambert G, et al. Femtosecond X rays from laser-plasma accelerators[J]. Reviews of Modern Physics, 2013, 85(1): 1-48.
[14] Faure J, Glinec Y, Pukhov A, et al. A laser-plasma accelerator producing monoenergetic electron beams[J]. Nature, 2004, 431(7008): 541-544.
[15] Geddes C G R, Toth C, van Tilborg J, et al. High-quality electron beams from a laser wakefield accelerator using plasma-channel guiding[J]. Nature, 2004, 431(7008): 538-541.
[16] Mangles S P D, Murphy C D, Najmudin Z, et al. Monoenergetic beams of relativistic electrons from intense laser-plasma interactions[J]. Nature, 2004, 431(7008): 535-538.
[17] Gonsalves A J, Nakamura K, Daniels J, et al. Petawatt laser guiding and electron beam acceleration to 8 GeV in a laser-heated capillary discharge waveguide[J]. Physical Review Letters, 2019, 122(8): 084801.
[18] Wang W T, Li W T, Liu J S, et al. High-brightness high-energy electron beams from a laser wakefield accelerator via energy chirp control[J]. Physical Review Letters, 2016, 117(12): 124801.
[19] Cipiccia S, Islam M R, Ersfeld B, et al. Gamma-rays from harmonically resonant betatron oscillations in a plasma wake[J]. Nature Physics, 2011, 7(11): 867-871.
[20] Chen L M, Yan W C, Li D Z, et al. Bright betatron X-ray radiation from a laser-driven-clustering gas target[J]. Scientific Reports, 2013, 3: 1912.
[21] Chen M, Luo J, Li F Y, et al. Tunable synchrotron-like radiation from centimeter scale plasma channels[J]. Light: Science & Applications, 2016, 5(1): e16015.
[22] Yu C H, Liu J S, Wang W T, et al. Enhanced betatron radiation by steering a laser-driven plasma wakefield with a tilted shock front[J]. Applied Physics Letters, 2018, 112(13): 133503.
[23] Phuoc K T, Corde S, Thaury C, et al. All-optical compton gamma-ray source[J]. Nature Photonics, 2012, 6(5): 308-311.
[24] Yu C H, Qi R, Wang W T, et al. Ultrahigh brilliance quasi-monochromatic MeV γ-rays based on self-synchronized all-optical Compton scattering[J]. Scientific Reports, 2016, 6: 29518.
[25] He Z H, Beaurepaire B, Nees J A, et al. Capturing structural dynamics in crystalline silicon using chirped electrons from a laser wakefield accelerator[J]. Scientific Reports, 2016, 6: 36224.
[26] Joshi C. Plasma-based accelerators: then and now[J]. Plasma Physics and Controlled Fusion, 2019, 61(10): 104001.
[27] Bulanov S V, Pegoraro F, Pukhov A M, et al. Transverse-wake wave breaking[J]. Physical Review Letters, 1997, 78(22): 4205-4208.
[28] Pukhov A, Meyer-ter-Vehn J. Laser wake field acceleration: the highly non-linear broken-wave regime[J]. Applied Physics B, 2002, 74(4/5): 355-361.
[29] Joshi C. Plasma accelerators[J]. Scientific American, 2006, 294(2): 40-47.
[30] Bulanov S, Naumova N, Pegoraro F, et al. Particle injection into the wave acceleration phase due to nonlinear wake wave breaking[J]. Physical Review E, 1998, 58(5): R5257-R5260.
[31] Kneip S, Nagel S R, Martins S F, et al. Near-GeV acceleration of electrons by a nonlinear plasma wave driven by a self-guided laser pulse[J]. Physical Review Letters, 2009, 103(3): 035002.
[32] Kostyukov I, Nerush E, Pukhov A, et al. Electron self-injection in multidimensional relativistic-plasma wake fields[J]. Physical Review Letters, 2009, 103(17): 175003.
[33] Umstadter D, Chen S Y, Maksimchuk A, et al. Nonlinear optics in relativistic plasmas and laser wake field acceleration of electrons[J]. Science, 1996, 273(5274): 472-475.
[34] Thomas A G R, Murphy C D, Mangles S P D, et al. Monoenergetic electronic beam production using dual collinear laser pulses[J]. Physical Review Letters, 2008, 100(25): 255002.
[35] Davoine X, Lefebvre E, Rechatin C, et al. Cold optical injection producing monoenergetic, multi-GeV electron bunches[J]. Physical Review Letters, 2009, 102(6): 065001.
[36] Oz E, Deng S, Katsouleas T, et al. Ionization-induced electron trapping in ultrarelativistic plasma wakes[J]. Physical Review Letters, 2007, 98(8): 084801.
[37] Pak A, Marsh K A, Martins S F, et al. Injection and trapping of tunnel-ionized electrons into laser-produced wakes[J]. Physical Review Letters, 2010, 104(2): 025003.
[38] Clayton C E, Ralph J E, Albert F, et al. Self-guided laser wakefield acceleration beyond 1 GeV using ionization-induced injection[J]. Physical Review Letters, 2010, 105(10): 105003.
[39] McGuffey C, Thomas A G R, Schumaker W, et al. Ionization induced trapping in a laser wakefield accelerator[J]. Physical Review Letters, 2010, 104(2): 025004.
[40] Liu J S, Xia C Q, Wang W T, et al. All-optical cascaded laser wakefield accelerator using ionization-induced injection[J]. Physical Review Letters, 2011, 107(3): 035001.
[41] Pollock B B, Clayton C E, Ralph J E, et al. Demonstration of a narrow energy spread, ∼0.5 GeV electron beam from a two-stage laser wakefield accelerator[J]. Physical Review Letters, 2011, 107(4): 045001.
[42] Suk H, Barov N, Rosenzweig J B, et al. Plasma electron trapping and acceleration in a plasma wake field using a density transition[J]. Physical Review Letters, 2001, 86(6): 1011-1014.
[43] Chien T Y, Chang C L, Lee C H, et al. Spatially localized self-injection of electrons in a self-modulated laser-wakefield accelerator by using a laser-induced transient density ramp[J]. Physical Review Letters, 2005, 94(11): 115003.
[44] Buck A, Wenz J, Xu J, et al. Shock-front injector for high-quality laser-plasma acceleration[J]. Physical Review Letters, 2013, 110(18): 185006.
[45] Rittershofer W, Schroeder C B, Esarey E, et al. Tapered plasma channels to phase-lock accelerating and focusing forces in laser-plasma accelerators[J]. Physics of Plasmas, 2010, 17(6): 063104.
[46] Swanson K K, Tsai H E, Barber S K, et al. Control of tunable, monoenergetic laser-plasma-accelerated electron beams using a shock-induced density downramp injector[J]. Physical Review Accelerators and Beams, 2017, 20(5): 051301.
[47] Ke L T, Yu C H, Feng K, et al. Optimization of electron beams based on plasma-density modulation in a laser-driven wakefield accelerator[J]. Applied Sciences, 2021, 11(6): 2560.
[48] Froula D H, Clayton C E, Döppner T, et al. Measurements of the critical power for self-injection of electrons in a laser wakefield accelerator[J]. Physical Review Letters, 2009, 103(21): 215006.
[49] Gonsalves A J, Nakamura K, Lin C, et al. Tunable laser plasma accelerator based on longitudinal density tailoring[J]. Nature Physics, 2011, 7(11): 862-866.
[50] Corde S, Thaury C, Lifschitz A, et al. Observation of longitudinal and transverse self-injections in laser-plasma accelerators[J]. Nature Communications, 2013, 4: 1501.
[51] Kuschel S, Schwab M B, Yeung M, et al. Controlling the self-injection threshold in laser wakefield accelerators[J]. Physical Review Letters, 2018, 121(15): 154801.
[52] Bloom M S, Streeter M J V, Kneip S, et al. Bright X-ray radiation from plasma bubbles in an evolving laser wakefield accelerator[J]. Physical Review Accelerators and Beams, 2020, 23(6): 061301.
[53] Faure J, Rechatin C, Norlin A, et al. Controlled injection and acceleration of electrons in plasma wakefields by colliding laser pulses[J]. Nature, 2006, 444(7120): 737-739.
[54] Rechatin C, Faure J, Ben-Ismail A, et al. Controlling the phase-space volume of injected electrons in a laser-plasma accelerator[J]. Physical Review Letters, 2009, 102(16): 164801.
[55] Golovin G, Yan W C, Luo J, et al. Electron trapping from interactions between laser-driven relativistic plasma waves[J]. Physical Review Letters, 2018, 121(10): 104801.
[56] Wenz J, Döpp A, Khrennikov K, et al. Dual-energy electron beams from a compact laser-driven accelerator[J]. Nature Photonics, 2019, 13(4): 263-269.
[57] Mo M Z, Ali A, Fourmaux S, et al. Quasimonoenergetic electron beams from laser wakefield acceleration in pure nitrogen[J]. Applied Physics Letters, 2012, 100(7): 074101.
[58] Mirzaie M, Li S, Zeng M, et al. Demonstration of self-truncated ionization injection for GeV electron beams[J]. Scientific Reports, 2015, 5: 14659.
[59] Maier A R, Delbos N M, Eichner T, et al. Decoding sources of energy variability in a laser-plasma accelerator[J]. Physical Review X, 2020, 10(3): 031039.
[60] Kirchen M, Jalas S, Messner P, et al. Optimal beam loading in a laser-plasma accelerator[J]. Physical Review Letters, 2021, 126(17): 174801.
[61] Wan Y, Seemann O, Tata S, et al. Direct observation of relativistic broken plasma waves[J]. Nature Physics, 2022, 18(10): 1186-1190.
[62] von der Leyen M W, Holloway J, Ma Y, et al. Observation of monoenergetic electrons from two-pulse ionization injection in quasilinear laser wakefields[J]. Physical Review Letters, 2023, 130(10): 105002.
[63] Geddes C G R, Nakamura K, Plateau G R, et al. Plasma-density-gradient injection of low absolute-momentum-spread electron bunches[J]. Physical Review Letters, 2008, 100(21): 215004.
[64] Schmid K, Buck A, Sears C M S, et al. Density-transition based electron injector for laser driven wakefield accelerators[J]. Physical Review Special Topics-Accelerators and Beams, 2010, 13(9): 091301.
[65] Ke L T, Feng K, Wang W T, et al. Near-GeV electron beams at a few per-mille level from a laser wakefield accelerator via density-tailored plasma[J]. Physical Review Letters, 2021, 126(21): 214801.
[66] Oubrerie K, Leblanc A, Kononenko O, et al. Controlled acceleration of GeV electron beams in an all-optical plasma waveguide[J]. Light: Science & Applications, 2022, 11: 180.
[67] Lu W, Tzoufras M, Joshi C, et al. Generating multi-GeV electron bunches using single stage laser wakefield acceleration in a 3D nonlinear regime[J]. Physical Review Special Topics - Accelerators and Beams, 2007, 10(6): 061301.
[68] Wang X M, Zgadzaj R, Fazel N, et al. Quasi-monoenergetic laser-plasma acceleration of electrons to 2 GeV[J]. Nature Communications, 2013, 4: 1988.
[69] Leemans W P, Gonsalves A J, Mao H S, et al. Multi-GeV electron beams from capillary-discharge-guided subpetawatt laser pulses in the self-trapping regime[J]. Physical Review Letters, 2014, 113(24): 245002.
[70] Qin Z Y, Li W T, Liu J Q, et al. Multi-GeV cascaded laser wakefield acceleration in a hybrid capillary discharge waveguide[J]. New Journal of Physics, 2022, 24(7): 073048.
[71] Zhu X Z, Li B Y, Liu F, et al. Experimental demonstration of laser guiding and wakefield acceleration in a curved plasma channel[J]. Physical Review Letters, 2023, 130(21): 215001.
[72] Litos M, Adli E, An W, et al. High-efficiency acceleration of an electron beam in a plasma wakefield accelerator[J]. Nature, 2014, 515(7525): 92-95.
[73] Emma P, Venturini M, Bane K L F, et al. Experimental demonstration of energy-chirp control in relativistic electron bunches using a corrugated pipe[J]. Physical Review Letters, 2014, 112(3): 034801.
[74] Brinkmann R, Delbos N, Dornmair I, et al. Chirp mitigation of plasma-accelerated beams by a modulated plasma density[J]. Physical Review Letters, 2017, 118(21): 214801.
[75] Döpp A, Thaury C, Guillaume E, et al. Energy-chirp compensation in a laser wakefield accelerator[J]. Physical Review Letters, 2018, 121(7): 074802.
[76] D’Arcy R, Wesch S, Aschikhin A, et al. Tunable plasma-based energy dechirper[J]. Physical Review Letters, 2019, 122(3): 034801.
[77] Wu Y P, Hua J F, Zhou Z, et al. Phase space dynamics of a plasma wakefield dechirper for energy spread reduction[J]. Physical Review Letters, 2019, 122(20): 204804.
[78] Zhang Z J, Li W T, Liu J S, et al. Energy spread minimization in a cascaded laser wakefield accelerator via velocity bunching[J]. Physics of Plasmas, 2016, 23(5): 053106.
[79] Di Mitri S, Cornacchia M. Electron beam brightness in linac drivers for free-electron-lasers[J]. Physics Reports, 2014, 539(1): 1-48.
[80] Lundh O, Lim J, Rechatin C, et al. Few femtosecond, few kiloampere electron bunch produced by a laser-plasma accelerator[J]. Nature Physics, 2011, 7(3): 219-222.
[81] Buck A, Nicolai M, Schmid K, et al. Real-time observation of laser-driven electron acceleration[J]. Nature Physics, 2011, 7(7): 543-548.
[82] Lehe R, Kirchen M, Andriyash I A, et al. A spectral, quasi-cylindrical and dispersion-free Particle-In-Cell algorithm[J]. Computer Physics Communications, 2016, 203: 66-82.
[83] Jalas S, Dornmair I, Lehe R, et al. Accurate modeling of plasma acceleration with arbitrary order pseudo-spectral particle-in-cell methods[J]. Physics of Plasmas, 2017, 24(3): 033115.
[84] Pousa A F, de la Ossa A M, Assmann R W. Intrinsic energy spread and bunch length growth in plasma-based accelerators due to betatron motion[J]. Scientific Reports, 2019, 9: 17690.
[85] Malaca B, Pardal M, Ramsey D, et al. Coherence and superradiance from a plasma-based quasiparticle accelerator[J]. Nature Photonics, 2024, 18: 39-45.
[86] Peng H, Huang T W, Jiang K, et al. Coherent subcycle optical shock from a superluminal plasma wake[J]. Physical Review Letters, 2023, 131(14): 145003.
[87] Rousse A, Phuoc K T, Shah R, et al. Production of a keV X-ray beam from synchrotron radiation in relativistic laser-plasma interaction[J]. Physical Review Letters, 2004, 93(13): 135005.
[88] Yan W C, Chen L M, Li D Z, et al. Concurrence of monoenergetic electron beams and bright X-rays from an evolving laser-plasma bubble[J]. Proceedings of the National Academy of Sciences of the United States of America, 2014, 111(16): 5825-5830.
[89] Ferri J, Corde S, Döpp A, et al. High-brilliance betatron γ-ray source powered by laser-accelerated electrons[J]. Physical Review Letters, 2018, 120(25): 254802.
[90] Guo B, Cheng Z, Liu S, et al. Enhancement of laser-driven betatron X-rays by a density-depressed plasma structure[J]. Plasma Physics and Controlled Fusion, 2019, 61(3): 035003.
[91] Zhu X L, Chen M, Weng S M, et al. Extremely brilliant GeV γ‑rays from a two-stage laser-plasma accelerator[J]. Science Advances, 2020, 6(22): eaaz7240.
[92] Popp A, Vieira J, Osterhoff J, et al. All-optical steering of laser-wakefield-accelerated electron beams[J]. Physical Review Letters, 2010, 105(21): 215001.
[93] Döpp A, Hehn L, Götzfried J, et al. Quick X-ray microtomography using a laser-driven betatron source[J]. Optica, 2018, 5(2): 199.
[94] Shou Y R, Wang P J, Lee S G, et al. Brilliant femtosecond-laser-driven hard X-ray flashes from carbon nanotube plasma[J]. Nature Photonics, 2023, 17(2): 137-142.
[95] Kozlova M, Andriyash I, Gautier J, et al. Hard X rays from laser-wakefield accelerators in density tailored plasmas[J]. Physical Review X, 2020, 10(1): 011061.
[96] Plateau G R, Geddes C G R, Thorn D B, et al. Low-emittance electron bunches from a laser-plasma accelerator measured using single-shot X-ray spectroscopy[J]. Physical Review Letters, 2012, 109(6): 064802.
[97] Qin Z Y, Yu C H, Wang W T, et al. Ultralow-emittance measurement of high-quality electron beams from a laser wakefield accelerator[J]. Physics of Plasmas, 2018, 25(2): 023106.
[98] Schwoerer H, Liesfeld B, Schlenvoigt H P, et al. Thomson-backscattered X rays from laser-accelerated electrons[J]. Physical Review Letters, 2006, 96(1): 014802.
[99] Chen S, Powers N D, Ghebregziabher I, et al. MeV-energy X rays from inverse Compton scattering with laser-wakefield accelerated electrons[J]. Physical Review Letters, 2013, 110(15): 155003.
[100] Powers N D, Ghebregziabher I, Golovin G, et al. Quasi-monoenergetic and tunable X-rays from a laser-driven Compton light source[J]. Nature Photonics, 2014, 8(1): 28-31.
[101] Liu C, Golovin G, Chen S Y, et al. Generation of 9 MeV γ-rays by all-laser-driven Compton scattering with second-harmonic laser light[J]. Optics Letters, 2014, 39(14): 4132-4135.
[102] Sarri G, Corvan D J, Schumaker W, et al. Ultrahigh brilliance multi-MeV γ‑ray beams from nonlinear relativistic Thomson scattering[J]. Physical Review Letters, 2014, 113(22): 224801.
[103] Yan W C, Fruhling C, Golovin G, et al. High-order multiphoton Thomson scattering[J]. Nature Photonics, 2017, 11(8): 514-520.
[104] Tsai H E, Wang X M, Shaw J M, et al. Compact tunable Compton X-ray source from laser-plasma accelerator and plasma mirror[J]. Physics of Plasmas, 2015, 22(2): 023106.
[105] Feng J, Wang J G, Li Y F, et al. Intense γ ray generated by refocusing laser pulse on wakefield accelerated electrons[J]. Physics of Plasmas, 2017, 24(9): 093110.
[106] Ride S K, Esarey E, Baine M. Thomson scattering of intense lasers from electron beams at arbitrary interaction angles[J]. Physical Review E, 1995, 52(5): 5425-5442.
[107] 赵振堂, 王东, 殷立新, 等. X射线自由电子激光试验装置[J]. 光学学报, 2021, 41(1): 0114006.
[108] Bostedt C, Boutet S, Fritz D M, et al. Linac Coherent Light Source: the first five years[J]. Reviews of Modern Physics, 2016, 88(1): 015007.
[109] Fuchs M, Weingartner R, Popp A, et al. Laser-driven soft-X-ray undulator source[J]. Nature Physics, 2009, 5(11): 826-829.
[110] Wang W T, Feng K, Ke L T, et al. Free-electron lasing at 27 nanometres based on a laser wakefield accelerator[J]. Nature, 2021, 595(7868): 516-520.
[111] Pompili R, Alesini D, Anania M P, et al. Free-electron lasing with compact beam-driven plasma wakefield accelerator[J]. Nature, 2022, 605(7911): 659-662.
[112] Labat M, Cabadağ J C, Ghaith A, et al. Seeded free-electron laser driven by a compact laser plasma accelerator[J]. Nature Photonics, 2023, 17(2): 150-156.
[113] Habib A F, Manahan G G, Scherkl P, et al. Attosecond-Angstrom free-electron-laser towards the cold beam limit[J]. Nature Communications, 2023, 14: 1054.
[114] 王岩成, 曹宗威, 孙向阳, 等. 激光驱动尾波场加速电子诱导光核嬗变[J]. 强激光与粒子束, 2023, 35(9): 091006.
Article Outline
余昌海, 秦志勇, 张志钧, 刘建胜. 激光尾波场电子加速及新型辐射源(特邀)[J]. 中国激光, 2024, 51(1): 0101002. Changhai Yu, Zhiyong Qin, Zhijun Zhang, Jiansheng Liu. Laser Wakefield Electron Acceleration and Novel Radiation Sources (Invited)[J]. Chinese Journal of Lasers, 2024, 51(1): 0101002.